![]()
Overview
Exercise represents one the highest levels of extreme stresses to which the body can be exposed. For example, in a person who has an extremely high fever approaching the level of lethality, the body metabolism increases to approximately 100% above normal; by comparison, the metabolism of the body during a marathon race increases to 2000% above normal.[1, 2, 3]
The following illustration depicts the cell structures of the skeletal muscle.
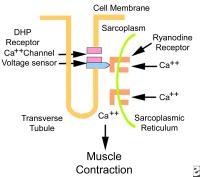
Excitation contraction coupling. DHP is dihydropyridine.
This article describes the basic physiology of exercise. The focus of this article is mainly at a subspecialty level; however, more detailed descriptions of various basic mechanisms are also provided for the casual reader.
For excellent patient education resources, visit eMedicineHealth's Healthy Living Center. Also, see eMedicineHealth's patient education articles Walking for Fitness and Resistance Training.
NextBasic Concepts -- Sex Differences
In general, the exercise-related measurements established for women follow the same general principles as those established for men, except for the quantitative differences caused by differences in body size, body composition, and levels of testosterone.
In women, the values of muscle strength, pulmonary ventilation, and cardiac output (all variables related with muscle mass) are generally 60-75% of the exercise physiology values recorded in men. When measured in terms of strength per square centimeter, the female muscle can achieve the same force of contraction as that of a male.[4, 5]
PreviousNextMusculoskeletal SystemFunctions of Muscle Tissue
Muscle tissue has 4 characteristics[1, 2, 3] that assume roles in homeostasis, as follows:
Excitability â Property of receiving and responding to stimuli such as the following: Neurotransmitters: Acetylcholine (ACh) stimulates skeletal muscle to contract.Electrical stimuli: Applying electrical stimuli between cardiac and smooth muscle cells causes the muscles to contract. Applying a shock to skeletal muscle causes contraction. Hormonal stimuli: Oxytocin stimulates smooth muscle in the uterus to contract during labor.Contractility â Ability to shortenExtensibility â Ability to stretch without damageElasticity â Ability to return to original shape after extension
Through contraction, muscle provides motion of the body (skeletal muscle), motion of blood (cardiac muscle), and motion of hollow organs such as the uterus, esophagus, stomach, intestines, and bladder (smooth muscle).
Muscle tissue also helps maintain posture and produce heat. A large amount of body heat is produced by metabolism and by muscle contraction. Muscle contraction during shivering warms the body.
Histology of Skeletal Muscle Tissue
Skeletal muscle consists of fibers (cells).[1, 2, 3] These cells are up to 100 µm in diameter and often are as long as the muscle. Each contains sarcoplasm (cytoplasm) and multiple peripheral nuclei per fiber. Skeletal muscle is actually formed by the fusion of hundreds of embryonic cells. Other cell structures (see image below) include the following:
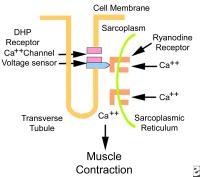
Excitation contraction coupling. DHP is dihydropyridine. Each fiber is covered by a sarcolemma (plasma membrane).The sarcoplasmic reticulum (smooth endoplasmic reticulum) stores calcium, which is released into the sarcoplasm during muscle contraction. Transverse tubules (T tubules), which are extensions of the sarcolemma that penetrate cells, transmit electrical impulses from the sarcolemma inward, so electrical impulses penetrate deeply into the cell. Besides conducting electricity along their walls, T tubules contain extracellular fluid rich in glucose and oxygen. The sarcoplasm of fiber is rich in glycogen (glucose polymer) granules and myoglobin (oxygen-storing protein). It also is rich in mitochondria.
Each fiber contains hundreds to thousands of rodlike myofibrils, which are bundles of thin and thick protein chains termed myofilaments. From a cross-sectional view of a myofibril, each thick filament is surrounded by a hexagonal array of 6 thin filaments. Each thin filament is surrounded by a triangular array of thick filaments.
Thin myofilaments are composed of 3 proteins: actin, tropomyosin, and troponin (see image below).
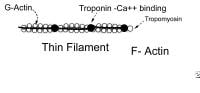
Thin filament. Thick myofilaments consist of bundles of approximately 200 myosin molecules (see image below). Myosin molecules look like double-headed golf clubs (both heads at the same end). The heads of the golf clubs are called myosin heads; they are also called cross-bridges because they link thick and thin filaments during contraction. They contain actin and adenosine triphosphate (ATP) binding sites. Myosin heads project out from the thick filaments, allowing them to bind to the thin filaments during contraction.
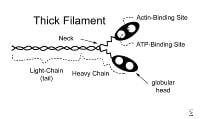
Thick filament. Actin is a long chain of multiple globular proteins, similar in shape to kidney beans. Each globular subunit contains a myosin-binding site. Tropomyosin is a long strand of protein that covers the myosin-binding sites on actin when the muscle is relaxed.Troponin is a polypeptide complex that binds to tropomyosin, helping to position it over the myosin-binding sites on actin. During muscle contraction, calcium binds troponin, which causes tropomyosin to roll off of the myosin binding sites on actin.
Muscle contraction (overview of the sliding filament mechanism)
A muscle action potential travels over sarcolemma and enters the T tubules, causing the sarcoplasmic reticulum to release calcium into the sarcoplasm. This triggers the contractile process.
Myosin cross-bridges pull on the actin myofilaments, causing the thin myofilaments of a sarcomere to slide toward the centers of the H zones, as shown below.
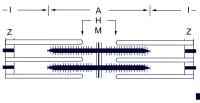
Sarcomere. Other Components of Skeletal Muscle
Connective-tissue components
Deep fascia is a broad band of dense irregular connective tissue beneath and around muscle and organs.[1, 2, 3] Deep fascia is different from superficial fascia, which is loose areolar connective tissue.
Other connective-tissue components (all are extensions of deep fascia) include epimysium, which covers the entire muscle; perimysium, which penetrates into muscle and surrounds bundles of fibers called fascicles; and endomysium, which is delicate, barely visible, loose areolar tissue covering individual fibers (ie, individual cells).
Tendons and aponeuroses are tough extensions of epimysium, perimysium, and endomysium. Tendons and aponeuroses are made of dense regular connective tissue and attach the muscle to bone or other muscle. Aponeuroses are broad, flat tendons. Tendon sheaths contain synovial fluid and enclose certain tendons. Tendon sheaths allow tendons to slide back and forth next to each other with lower friction. Tenosynovitis is inflammation of the tendon sheaths and tendons, especially those of the wrists, shoulders, and elbows. Tendons are not contractile and not very stretchy; furthermore, they are not very vascular and they heal poorly.
Nerve and blood supply
Nerves convey impulses for muscular contraction. Nerves are bundles of nerve cell processes. Each nerve cell process (ie, axon) divides at its tip into a few to 10,000 branches called telodendria. At the end of each of these branches is an axon terminal that is rich in neurotransmitters.
Blood provides nutrients and oxygen for contraction. An artery and a vein usually accompany a nerve that penetrates skeletal muscle. Arteries in muscles dilate during active muscular activity, thus increasing the supply of oxygen and glucose.
Motor units
A motor nerve is a bundle of axons that conducts nerve impulses away from the brain or spinal cord toward muscles. Each axon transmits an action potential (ie, nerve impulse), which is a burst of electricity. The nerve impulse travels along the axons at a steady rate, like fire travels along a fuse; however, nerve impulses travel extremely fast. Each axon has 4-2000 or more branches (ie, telodendria), with an average of 150 telodendria. Each separate branch supplies a separate muscle cell. Thus, if an axon has 10 branches, it supplies 10 muscle fibers. Small motor units are for fine control of muscles; large motor units are for muscles that do not require such fine control.
Neuromuscular junction
The neuromuscular junction is made of an axon terminal and the portion of the muscle fiber sarcolemma it nearly touches (called the motor endplate). The neurotransmitter released at the neuromuscular junction in skeletal muscle is ACh. The motor endplate is rich in thousands of ACh receptors; the receptors are integral proteins containing binding sites for ACh and sodium channels.
Physiology of ContractionNerve impulse (action potential) reaches the axon terminal, which triggers calcium influx into the axon terminal.[1, 2, 3] Calcium influx causes synaptic vesicles to release ACh via exocytosis.ACh diffuses across synaptic cleft.ACh binds to the ACh receptor on the sarcolemma. Succinylcholine, a drug used to induce paralysis during surgery, binds to ACh receptors more tightly than ACh. Succinylcholine initially causes some depolarization, but then it binds to the receptor, preventing ACh from binding. Therefore, it blocks the muscle's stimulation by ACh, causing paralysis. Another drug that acts in a similar fashion is curare. These drugs do not cause pain relief or unconsciousness; thus, they are combined with other drugs during surgery. When ACh binds the receptor, it opens chemically regulated ion channels, which are sodium channels through the receptor molecule. Sodium, which is in high concentration outside cells and in low concentration inside cells, rushes into the cell through the channels. The cell, whose resting membrane potential along the inside of the membrane is negative when compared with the outside of the membrane, becomes positively charged along the inside of the membrane when sodium (a positive ion) rushes in. This change from a negative charge to a positive charge along the inner membrane is termed depolarization. The depolarization of one region of the sarcolemma (the motor endplate) initiates an action potential, which is a propagating wave of depolarization that travels (propagates) along the sarcolemma. Regions of membrane that become depolarized rapidly restore their proper ionic concentrations along their inner and outer surfaces in a process termed repolarization. (This process of depolarization, propagation, and repolarization is similar to dominoes that topple each other but also spring back into the upright position shortly afterward.) The action potential also propagates along the membrane lining the T tubules entering the cell.This action potential traveling along the T tubules causes the sarcoplasmic reticulum to release calcium into sarcoplasm.Calcium binds with troponin, causing it to pull on tropomyosin to change its orientation, exposing myosin-binding sites on actin. An ATPase, which also functions as a myosin cross-bridging protein, splits ATP into adenosine diphosphate (ADP) + phosphate (P) in the previous contraction cycle. This energizes the myosin head. The energized myosin head, or cross-bridge, combines with myosin-binding sites on actin. Power stroke occurs. The attachment of the energized cross-bridge triggers a pivoting motion (ie, power stroke) of the myosin head. During the power stroke, ADP and P are released from the myosin cross-bridge. The power stroke causes thin actin myofilaments to slide past thick myosin myofilaments toward the center of the A bands (see the following image).
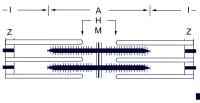
Sarcomere. ATP attaches to the myosin head again, allowing it to detach from actin. (In rigor mortis, an ATP deficiency occurs. Cross-bridges remain, and the muscles are rigid.) ATP is broken down to ADP and P, which cocks the myosin head again, preparing it to perform another power stroke if needed.Repeated detachment and reattachment of the cross-bridges results in shortening without much increase in tension during the shortening phase (isotonic contraction) or results in increased tension without shortening (isometric contraction). Release of the enzyme acetylcholinesterase in the neuromuscular junction destroys ACh and stops the generation of a muscle action potential. Calcium is taken back up (resequestered) in the sarcoplasmic reticulum, and myosin cross-bridges separate. ATP is required to separate myosin-actin cross-bridges. The muscle fiber resumes its resting state. Muscle Metabolic Systems During Exercise
The chemical energy that fuels muscular activities is ATP.[1, 2, 3] For the first 5 or 6 seconds of muscle power, muscular activity can depend on the ATP that is already present in the muscle cells. Beyond this time, new amounts of ATP must be formed to enable the activation of muscular contractions that are needed to support longer and more vigorous physical activities.
For activities that require a quick burst of energy that cannot be supplied by the ATP present in the muscle cells, the next 10-15 seconds of muscle power can be provided through the bodyâs use of the phosphagen system, which uses a substance called creatine phosphate to recycle ADP into ATP.[6] For longer and more intense periods of physical activity, the body must rely on systems that break down the sugars (glucose) to produce ATP.
The complete breakdown of glucose occurs in 2 ways: through anaerobic respiration (does not use oxygen) and through aerobic respiration (occurs in the presence of oxygen). The anaerobic use of glucose to form ATP occurs as the body increases its muscle use beyond the capability of the phosphagen system to supply energy. In particular, the glycogen lactic acid system, through its anaerobic breakdown of glucose, provides approximately 30-40 seconds more of maximal muscle activity. For this system, each glucose molecule is split into 2 pyruvic acid molecules, and energy is released to form several ATP molecules, providing the extra energy. Then, the pyruvic acid partially breaks down further to produce lactic acid. If the lactic acid is allowed to accumulate in the muscle, one experiences muscle fatigue. At this point, the aerobic system must activate.
The aerobic system in the body is used for sports that require an extensive and enduring expenditure of energy, such as a marathon race. Endurance sports absolutely require aerobic energy. A large amount of ATP must be provided to muscles to sustain the muscle power needed to perform such events without an excessive production of lactic acid. This can only be accomplished when oxygen in the body is used to break down the pyruvic acid (that was produced anaerobically) into carbon dioxide, water, and energy by way of a very complex series of reactions known as the citric acid cycle. This cycle supports muscle usage for as long as the nutrients in the body last.
The breakdown of pyruvic acid requires oxygen and slows or eliminates the accumulation of lactic acid. In summary, the 3 different muscle metabolic systems that supply the energy required for various activities are as follows:
Phosphagen system (for 10- to 15-sec bursts of energy)Glycogen lactic acid system (for another 30-40 sec of energy)Aerobic system (provides a great deal of energy that is only limited by the body's ability to supply oxygen and other important nutrients)
Many sports require the use of a combination of these metabolic systems. By considering the vigor of a sports activity and its duration, one can estimate very closely which of the energy systems are used for each activity.
Postexercise Recovery
Oxygen debt
During muscular exercise, blood vessels in muscles dilate and blood flow is increased in order to increase the available oxygen supply.[1, 2, 3] Up to a point, the available oxygen is sufficient to meet the energy needs of the body. However, when muscular exertion is very great, oxygen cannot be supplied to muscle fibers fast enough, and the aerobic breakdown of pyruvic acid cannot produce all the ATP required for further muscle contraction.
During such periods, additional ATP is generated by anaerobic glycolysis. In the process, most of the pyruvic acid produced is converted to lactic acid. Although approximately 80% of the lactic acid diffuses from the skeletal muscles and is transported to the liver for conversion back to glucose or glycogen, some lactic acid accumulates in muscle tissue, making muscle contraction painful and causing fatigue. Ultimately, once adequate oxygen is available, lactic acid must be catabolized completely into carbon dioxide and water.
After exercise has stopped, extra oxygen is required to metabolize lactic acid; to replenish ATP, phosphocreatine, and glycogen; and to replace (âpay backâ) any oxygen that has been borrowed from hemoglobin, myoglobin (an iron-containing substance similar to hemoglobin that is found in muscle fibers), air in the lungs, and body fluids. The additional oxygen that must be taken into the body after vigorous exercise to restore all systems to their normal states is called oxygen debt. The debt is paid back by labored breathing that continues after exercise has stopped. Thus, the accumulation of lactic acid causes hard breathing and sufficient discomfort to stop muscle activity until homeostasis is restored.[7]
Recovery of muscle glycogen postexercise
Eventually, muscle glycogen must also be restored. Restoration of muscle glycogen is accomplished through diet and may take several days, depending on the intensity of exercise. The maximum rate of oxygen consumption during the aerobic catabolism of pyruvic acid is called maximal oxygen uptake.
Maximal oxygen uptake is determined by sex (higher in males), age (highest at approximately age 20 y), and size (increases with body size). Highly trained athletes can have maximal oxygen uptakes that are twice that of average people, probably owing to a combination of genetics and training. As a result, highly trained athletes are capable of greater muscular activity without increasing their lactic acid production and have lower oxygen debts, which is why they do not become short of breath as readily as untrained individuals.
Fuel Usage
Fuel usage (light exercise)
The best examples of light exercise are walking and light jogging.[1, 2, 3] The muscles that are recruited during this type of exercise are those that contain a large amount of type I muscle cells, and, because these cells have a good blood supply, it is easy for fuels and oxygen to travel to the muscle. ATP consumption makes ADP available for new ATP synthesis.
The presence of ADP (and the resulting synthesis of ATP) simulates the movement of hydrogen (H+) into the mitochondria; this, in turn, reduces the proton gradient and thus stimulates electron transport. The hydrogen on the reduced form of nicotinamide adenine dinucleotide (NADH) is used up, nicotinamide adenine dinucleotide (NAD) becomes available, and fatty acids and glucose are oxidized.
Incidentally, the calcium released during contraction stimulates the enzymes in the Krebs cycle and stimulates the movement of the glucose transporter 4 (GLUT-4) from inside of the muscle cell to the cell membrane. Both these exercise-induced responses augment the elevation in fuel oxidation caused by the increase in ATP consumption.
Fuel usage (moderate exercise)
An increase in the pace of running simply results in an increased rate of fuel consumption, an increased fatty acid release, and, therefore, an increase in the rate of muscle fatty acid oxidation. However, if the intensity of the exercise increases even further, a stage is reached in which the rate of fatty acid oxidation becomes limited.
The reasons why the rate of fatty acid oxidation reaches a maximum are not clear, but it is possible that the enzymes in the beta-oxidation pathway are saturated (ie, they reach a stage in which their maximal velocity [Vmax] is less than the rate of acetyl-coenzyme A [acetyl-CoA] consumption in the Krebs cycle). Alternatively, it may be that the availability of carnitine (the chemical required to transport the fatty acids into the mitochondria) becomes limited.
Whatever the reason, the consequence is that as the pace rises, the demand for acetyl-CoA cannot be met by fatty acid oxidation alone. The accumulation of acetyl-CoA that was so effective at inhibiting the oxidation of glucose is no longer present, so pyruvate dehydrogenase starts working again and pyruvate is converted into acetyl-CoA. In other words, more of the glucose that enters the muscle cell is oxidized fully to carbon dioxide. Therefore, the energy used during moderate exercise is derived from a mixture of fatty acid and glucose oxidation.
Fuel usage (strenuous exercise)
As the intensity of the exercise increases even further (ie, running at the pace of middle-distance races), the rate at which the muscles can extract glucose from the blood becomes limited. In other words, the rate of glucose transport reaches Vmax, either because the blood cannot supply the glucose fast enough or the number of GLUT-4s becomes limited. ATP generation cannot be serviced completely by exogenous fuels, and ATP levels decrease. Not only does this stimulate phosphofructokinase, it also stimulates glycogen phosphorylase. This means that glycogen stored within the muscle cells is broken down to provide glucose. Therefore, the fuel mix during strenuous exercise is composed of contributions from blood-borne glucose and fatty acids and from endogenously stored glycogen.
Fuel usage in individuals who are unfit
Being fit (biochemically speaking) means that the individual has a well-developed cardiovascular system that can efficiently supply nutrients and oxygen to the muscles. Fit people have muscle cells that are well perfused with capillaries (ie, they have a good muscle blood supply). Their muscle cells also have a large number of mitochondria, and those mitochondria have a high activity of Krebs cycle enzymes, electron transport carriers, and oxidation enzymes.
Individuals who are unfit must endure the consequences of a poorer blood supply, fewer mitochondria, less electron transport units, a lower activity of the Krebs cycle, and poorer activity of beta-oxidation enzymes. To generate ATP in the mitochondria, a steady supply of fuel and oxygen and decent activity of the oxidizing enzymes and carriers are needed. If any of these components are lacking, the rate at which ATP can be produced by mitochondria is compromised. Under these circumstances, the production of ATP by aerobic means is not sufficient to provide the muscles with sufficient ATP to sustain contractions. The result is anaerobic ATP generation using glycolysis. Increasing the flux through glycolysis but not increasing the oxidative consumption of the resulting pyruvate increases the production of lactate.
PreviousNextPulmonary Physiology During Exercise
The purpose of respiration is to provide oxygen to the tissues and to remove carbon dioxide from the tissues.[1, 2, 3] To accomplish this, 4 major events must be regulated, as follows:
Pulmonary ventilationDiffusion of oxygen and carbon dioxide between the alveoli and the bloodTransport of oxygen and carbon dioxide in the blood and body fluids and to and from the cellsRegulation of ventilation and other aspects of respiration: Exercise causes these factors to change, but the body is designed to maintain homeostasis.
When one goes from a state of rest to a state of maximal intensity of exercise, oxygen consumption, carbon dioxide formation, and total pulmonary and alveolar ventilation increase by approximately 20-fold. A linear relationship exists between oxygen consumption and ventilation. At maximal exercise, pulmonary ventilation is 100-110 L/min, whereas maximal breathing capacity is 150-170 L/min. Thus, the maximal breathing capacity is approximately 50% greater than the actual pulmonary ventilation during maximal exercise. This extra ventilation provides an element of safety that can be called on if the situation demands it (eg, at high altitudes, under hot conditions, abnormality in the respiratory system). Therefore, the respiratory system itself is not usually the most limiting factor in the delivery of oxygen to the muscles during maximal muscle aerobic metabolism.
VO2max is the rate of oxygen consumption under maximal aerobic metabolism. This rate in short-term studies is found to increase only 10% with the effect of training. However, that of a person who runs in marathons is 45% greater than that of an untrained person. This is believed to be partly genetically determined (eg, stronger respiratory muscles, larger chest size in relation to body size) and partly due to long-term training.
Oxygen diffusing capacity is a measure of the rate at which oxygen can diffuse from the alveoli into the blood. An increase in diffusing capacity is observed in a state of maximal exercise. This results from the fact that blood flow through many of the pulmonary capillaries is sluggish in the resting state. In exercise, increased blood flow through the lungs causes all of the pulmonary capillaries to be perfused at their maximal level, providing a greater surface area through which oxygen can diffuse into the pulmonary capillary blood. Athletes who require greater amounts of oxygen per minute have been found to have higher diffusing capacities, but the exact reason why is not yet known. Although one would expect the oxygen pressure of arterial blood to decrease during strenuous exercise and carbon dioxide pressure of venous blood to increase far above normal, this is not the case. Both of these values remain close to normal.
Stimulatory impulses from higher centers of the brain and from joint and muscle proprioceptive stimulatory reflexes account for the nervous stimulation of the respiratory and vasomotor center that provides almost exactly the proper increase in pulmonary ventilation to keep the blood respiratory gases almost normal. If nervous signals are too strong or weak, chemical factors bring about the final adjustment in respiration that is required to maintain homeostasis.
PreviousNextCardiovascular System and Exercise
Regular exercise makes the cardiovascular system more efficient at pumping blood and delivering oxygen to the exercise muscles.[1, 2, 3, 8, 9, 10] Releases of adrenaline and lactic acid into the blood result in an increase of the heart rate (HR).
Basic definitions of terms are as follows:
VO2 equals cardiac output times oxygen uptake necessary to supply oxygen to muscles.The Fick equation is the basis for determination of VO2 (see image below).[10]
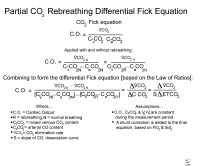
Fick equation.
Exercises increase some of the different components of the cardiovascular system, such as stroke volume (SV), cardiac output, systolic blood pressure (BP), and mean arterial pressure. A greater percentage of the cardiac output goes to the exercising muscles. At rest, muscles receive approximately 20% of the total blood flow, but during exercise, the blood flow to muscles increases to 80-85%.
To meet the metabolic demands of skeletal muscle during exercise, 2 major adjustments to blood flow must occur. First, cardiac output from the heart must increase. Second, blood flow from inactive organs and tissues must be redistributed to active skeletal muscle.
Generally, the longer the duration of exercise, the greater the role the cardiovascular system plays in metabolism and performance during the exercise bout. An example would be the 100-meter sprint (little or no cardiovascular involvement) versus a marathon (maximal cardiovascular involvement).
General functions of the cardiovascular system
The cardiovascular system helps transport oxygen and nutrients to tissues, transport carbon dioxide and other metabolites to the lungs and kidneys, and distribute hormones throughout the body. The cardiovascular system also assists with thermoregulation.
Cardiac cycle
The pumping of blood by the heart requires the following 2 mechanisms to be efficient:
Alternate periods of relaxation and contraction of the atria and ventriclesCoordinated opening and closing of the heart valves for unidirectional flow of blood
The cardiac cycle is divided into 2 phases: ventricular diastole and ventricular systole.
Ventricular diastole This phase begins with the opening of the atrioventricular (AV) valves. The mitral valve (located between the left atrium and left ventricle) opens when the left ventricular pressure falls below the left atrial pressure, and the blood from left atrium enters the left ventricle. Later, as the blood continues to flow into the left ventricle, the pressure in both chambers tends to equalize.At the end of the diastole, left atrial contractions cause an increase in left atrial pressure, thus again creating a pressure gradient between the left atrium and ventricle and forcing blood into the left ventricle. Ventricular systole Ventricular systole begins with the contraction of the left ventricle, which is caused by the spread of an action potential over the left ventricle. The contraction of the left ventricle causes an increase in the left ventricular pressure. When this pressure is higher than the left atrial pressure, the mitral valve is closed abruptly. The left ventricular pressure continues to rise after the mitral valve is closed. When the left ventricular pressure rises above the pressure in the aorta, the aortic valve opens. This period between the closure of the mitral valve and the opening of the aortic valve is called isovolumetric contraction phase. The blood ejects out of the left ventricle and into the aorta once the aortic valve is opened. As the left ventricular contraction is continued, 2 processes lead to a fall in the left ventricular pressure. These include a decrease in the strength of the ventricular contraction and a decrease in the volume of blood in the ventricle. When the left ventricular pressure falls below the aortic pressure, the aortic valve is closed. After the closure of the aortic valve, the left ventricular pressure falls rapidly as the left ventricle relaxes. When this pressure falls below the left atrial pressure, the mitral valve opens and allows blood to enter left ventricle. The period between the closure of the aortic valve closure and the opening of the mitral valve is called isovolumetric relaxation time. Right-sided heart chambers undergo the same phases simultaneously.Pressure changes during the cardiac cycle
Most of the work of the heart is completed when ventricular pressure exists. The greater the ventricular pressure, the greater the workload of the heart. Increases in BP dramatically increase the workload of the heart, and this is why hypertension is so harmful to the heart.
Arterial BP is the pressure that is exerted against the walls of the vascular system. BP is determined by cardiac output and peripheral resistance. Arterial pressure can be estimated using a sphygmomanometer and a stethoscope. The reference range for males is 120/80 mm Hg; the reference range for females is 110/70 mm Hg.
The difference between systolic and diastolic pressure is called the pulse pressure. The average pressure during a cardiac cycle is called the mean arterial pressure (MAP). MAP determines the rate of blood flow through the systemic circulation.
During rest, MAP = diastolic BP + (0.33 X pulse pressure). For example, MAP = 80 + (0.33 X [120-80]), MAP = 93 mm Hg.During exercise, MAP = diastolic BP + (0.50 X pulse pressure). For example, MAP = 80 + (0.50 X [160-80]), MAP = 120 mm Hg.Coordinated control of the heart
The heart has the ability to generate its own electrical activity, which is known as intrinsic rhythm. In the healthy heart, contraction is initiated in the sinoatrial (SA) node, which is often called the heart's pacemaker. If the SA node cannot set the rate, then other tissues in the heart are able to generate an electrical potential and establish the HR.
Control of cardiac output (HR)
The parasympathetic nervous system and the sympathetic nervous system affect a person's HR.
Parasympathetic nervous system: The vagus nerve originates in the medulla and innervates the SA and AV nodes. The nerve releases ACh as the neurotransmitter. The response is a decrease in SA node and AV node activity, which causes a decrease in HR. Sympathetic nervous system: The nerves arise from the spinal cord and innervate the SA node and ventricular muscle mass. The nerves release norepinephrine as the neurotransmitter. The response is an increase in HR and a force of contraction of the ventricles. Control of sympathetic and parasympathetic activity
At rest, sympathetic and parasympathetic nervous stimulation are in balance. During exercise, parasympathetic stimulation decreases and sympathetic stimulation increases. Several factors can alter sympathetic nervous system input.
Baroreceptors are groups of neurons located in the carotid arteries, the arch of aorta, and the right atrium. These neurons sense changes in pressure in the vascular system. An increase in BP results in an increase in parasympathetic activity except during exercise, when the sympathetic activity overrides the parasympathetic activity.
Chemoreceptors are groups of neurons located in the arch of the aorta and the carotid arteries. These neurons sense changes in oxygen concentration. When oxygen concentration in the blood is decreased, parasympathetic activity decreases and sympathetic activity increases.
Temperature receptors are neurons located throughout the body. These neurons are sensitive to changes in body temperature. As temperature increases, sympathetic activity increases to cool the body and to reduce internal core temperature.
Control of cardiac output (SV)
SV is controlled by end-diastolic volume, average aortic BP, and the strength of ventricular contraction.
End-diastolic volume: This is often referred to as the preload. If the end-diastolic volume increases, the SV increases. With an increased end-diastolic volume, a slight stretching of the cardiac muscle fibers occurs, which increases the force of contraction Average aortic BP: This is often referred to as the afterload. The BP in the aorta represents a barrier to the blood being ejected from the heart. The SV is inversely proportional to the aortic BP. During exercise, the afterload is reduced, which allows for an increase in SV. Strength of ventricular contraction: Epinephrine and norepinephrine can increase the contractility of the heart by increasing the calcium concentration within the cardiac muscle fiber. Epinephrine and norepinephrine allow for greater calcium entry through the calcium channels in cardiac muscle fiber membranes. This allows for greater myosin and actin interaction and an increase in force production. Control of cardiac output (venous return)
Venoconstriction occurs as a response to sympathetic nervous system stimulation. Sympathetic stimulation constricts the veins that drain skeletal muscle. This causes greater blood to flow back to the heart.
The muscle pump is the rhythmic contraction and relaxation of skeletal muscle that compresses the veins and thus drains the skeletal muscle. This causes greater blood flow back to the heart. The muscle pump is very important during both resting and exercise conditions.
During exercise, the respiratory pump helps increase venous return. The pressure within the chest decreases and abdominal pressure increases with inhalation, thus facilitating blood flow back to the heart. Because of the increased respiratory rate and depth of breathing during exercise, this is an effective way to increase venous return.
Hemodynamics
The circulatory system is a closed-loop system, and flow through the circulatory system is the result of pressure differences between the 2 ends of the system, the left ventricle (90 mm Hg) and the right atrium (approximately 0 mm Hg).
Systemic blood flow affects hemodynamics. The control of blood flow during exercise is extremely important to ensure that blood and oxygen are transported to the tissues that need them most. Blood flow to tissues is dependent on the relationship between BP and the resistance provided by the blood vessels.
Blood flow at rest is equal to the change in pressure divided by the resistance of the vessels (ie, BF = P/R, where BF is blood flow, P is pressure, and R is resistance). Blood flow during exercise is regulated by changing BP and altering the peripheral resistance of the vessels.
The pressure change at rest in the cardiovascular system is 93 mm Hg, as follows: Mean aortic pressure = 93 mm Hg, mean right atrial pressure = 0 mm Hg, and driving pressure in the system = 93 mm Hg.
During exercise, BP increases so that blood flow through the body increases. Blood flow is also increased during exercise by decreasing the resistance of the vessels in the systemic circulation of active skeletal muscle. Resistance is determined by the following formula:
Resistance = (length of tube X viscosity of blood)/radius4.
Changing the radius of the vessels has the most profound effect on blood flow. Doubling the radius of a blood vessel decreases resistance by a factor of 16. Decreasing the radius of a blood vessel by half increases resistance by a factor of 16. The arterioles have the most control over blood flow in the systemic circulation.
Changes in oxygen delivery to muscle during exerciseBP increases as exercise intensity increases, rising from approximately 120 mm Hg to approximately 200 mm Hg.SV increases during exercise until 40% of VO2max (maximum oxygen uptake level) is reached, rising from approximately 80 mL/beat to approximately 120 mL/beat.HR increases with intensity until VO2max is reached, rising from approximately 70 beats per minute to approximately 200 beats per minute.Cardiac output increases with intensity until VO2max is reached, rising from approximately 5 L/min to approximately 25-30 L/min.
The arterial-venous oxygen difference is the amount of oxygen extracted from the blood as it passes through the capillary bed. This difference rises from approximately 4 mL of oxygen per 100 mL of blood at rest to approximately 18 mL of oxygen per 100 mL of blood during high-intensity aerobic exercise.
Redistribution of blood flow during exercise
At rest, 15-20% of blood goes to skeletal muscle; during exercise, this amount increases to 80-85%. The percentage of blood to the brain decreases, but the absolute amount increases. The same percentage of blood goes to cardiac muscle, but the absolute amount increases. Blood flow to visceral tissues and inactive skeletal muscle reduces. In addition, the cutaneous blood flow initially decreases, but it later increases during the course of exercise.
The redistribution of the blood is brought about by several mechanisms. During exercise, generalized vasodilatation occurs because of the accumulation of vasodilatory metabolites. This leads to a decrease in the peripheral resistance, which, in turn, elicits a strong increase in the sympathetic activity through the activation of baroreceptors. The increase in sympathetic activity leads to vasoconstriction in the visceral organs, whereas the vasodilatation predominates in the blood vessels of the muscles and the coronary circulation because of the local vasodilatory metabolites. The cutaneous blood vessels initially respond to the sympathetic activity by vasoconstriction. As the exercise continues, temperature reflexes are activated and cause cutaneous vasodilatation to dissipate the heat produced by the muscle activity, resulting in an increase in the cutaneous blood flow.
Regulation of blood flow at the local level
The local blood flow is controlled by chemical factors, metabolites, paracrines, physical factors such as heat or cold, stretch effects on endothelial membrane, active hyperemia, and reactive hyperemia. The paracrine regulation is mainly regulated by nitric oxide, histamine release, and prostacyclin. Nitric oxide diffuses to smooth muscle and causes vasodilation by reducing calcium entry into smooth muscle.
Regulation of cardiovascular function
HR and blood flow are controlled by various centers in the brain. These centers receive input from receptors located throughout the body. The centers work to initiate the appropriate response from tissues and organs in the body.
Aerobic exercise requires oxygen to be present for the generation of energy from fuels such as glucose or glycogen. Aerobic exercise results in no buildup of lactic acid as a result of metabolism. This process is more efficient than anaerobic metabolism. During normal rest and aerobic exercise, carbohydrates and fats are used as fuels. A high degree of aerobic fitness requires a well-adapted ability to take in, carry, and use oxygen. Laboratory measurements are most accurate, but they are expensive. An individual's fitness level may be estimated according to these measurements.
Anaerobic exercise produces lactic acid and is usually of short duration. Anaerobic exercise is high intensity and has a greater inherent risk of injury. Individuals who are unfit have a lower anaerobic threshold than athletes who are aerobically trained. The well-trained athlete may be able to approach 80% of the VO2max aerobically without lactate production.
The usual VO2 measurements are in L/min; however, if the size of the individual needs to be accounted for, the measurements may be in mL/kg/min. The values for the average person aged 20 years are 37-48 mL/kg/min. Male athletes who are highly trained may approach measurements in the high 70s to low 80s. Training enhances the ability of the body, in particular the muscle cells, to better handle oxygen. Muscle must be able to use oxygen efficiently to keep anaerobic metabolism at a given level of effort to a minimum.
Cardiac output is a major determinant of oxygen uptake. VO2max declines with age as the maximum HR declines. This is one of the major factors causing the approximately 7% decline with each decade of life after age 30 years. Muscle training and use of oxygen at the end organ, muscle, is the second factor that affects oxygen uptake. The arterial-venous oxygen difference comes about as a combination of arterial oxygen content, shunting of blood to muscles, and the muscle extraction of oxygen.
Training results in a more efficient heart and an increase in the maximum SV. An increase in VO2 results in an ease in the stress of a given workload. When maximum SV is increased, the heart can work more efficiently at a given pulse rate. This lessens the necessity of an increased pulse at a given workload. Resting pulse is lower, as is the pulse at any given workload.
One metabolic unit (MET) equals the VO2 at rest. The estimate of the value of one MET is 3.5 mL of oxygen per kg/min. Conversion of VO2 measurements may be obtained by dividing the value of the VO2 in mL of oxygen per kg/min by the value of one MET or 3.5. For example, a VO2 measurement of 35 mL of oxygen per kg/min is equivalent to an output of 10 METs.
Cardiovascular changes with isometric exercise
Cardiovascular changes during isometric exercise differ from those during dynamic exercise. Static exercise causes compression of the blood vessels in the contracting muscles, leading to a reduction in the blood flow in them. Therefore, total peripheral resistance, which normally falls during dynamic exercise, does not fall and may, in fact, increase, especially if several large groups of muscles are involved in the exercise. The activation of the sympathetic system with exercise thus leads to an increase in HR, cardiac output, and BP.
Because the total peripheral resistance does not decrease, the increase in HR and cardiac output is less and an increase in the systolic, diastolic, and mean arterial pressure is more compared with those seen with dynamic exercise. Because BP is a major determinant of afterload, the left ventricular wall stress, and thus the cardiac workload, is significantly higher during static exercise compared with the cardiac workload achieved during dynamic exercise.
Cardiac changes following training
In most cases, the SV plateaus at a VO2 of approximately 40-60% of the maximum. This applies to both trained and untrained males and females. The SV for untrained males may approach 100-120 mL/beat/min. For trained males, this value is 150-170 mL/beat/min. For highly trained athletes, maximal SV may reach or even exceed 200 mL/beat/min. The values for women are lower than those for men. Maximal SV for untrained women is usually between 80 mL/beat/min, and for trained women, it is usually between 100 mL/beat/min. These changes translate into an increase in the circulation blood volume and in cardiac output, with a corresponding decrease in the resting HR and the resting and exercise BP.
The heart undergoes certain morphologic changes in response to chronic exercise, commonly seen via echocardiography. These morphologic changes define what is commonly referred to as an "athletic heart." Athletic heart syndrome is characterized by hypertrophy of the myocardium (ie, an increase in the mass of the myocardium).
Although the hypertrophy in athlete's heart is morphologically similar to that seen in patients with hypertension, several important differences exist. In contrast to the hypertension-induced hypertrophy, the hypertrophy in the athletic heart is noted in absence of any diastolic dysfunction, with a normal isovolumetric relaxation time, with no decrease in the peak rate of left ventricular filling, and with no decrease in the peak rate of left ventricular cavity enlargement and wall thinning. Because the wall stress in the athlete's heart is normal, sometimes the hypertrophy seems to be disproportionate to the level of resting BP.
In addition, the rate of decline in the left ventricular hypertrophy and mass is much more rapid when the training is stopped compared with the regression in the same parameters in treated hypertension. On average, the decline in these parameters is seen 3 weeks after stopping exercise, and these morphologic changes can be seen on echocardiograms.
Sometimes, these morphologic changes are confused with the changes seen in patients with hypertrophic cardiomyopathy (HCM). A few important morphologic differences exist. In athletic heart syndrome, the hypertrophy is usually symmetrical, as opposed to the asymmetrical hypertrophy in HCM. Also, the left ventricular size is generally normal or increased, and the left atrial size is normal, as opposed to a small left ventricular cavity with a larger left atrial cavity size (usually >4.5 cm) in HCM. Despite these differences, sometimes making a distinction between 2 conditions is a challenge.
PreviousNextSummary
In summary, exercise is accomplished by alteration in the body response to the physical stress (exercise physiology). These responses to exercise include an increase in the HR, BP, SV, cardiac output, ventilation, and VO2. The metabolism at the cellular level is also modulated to accommodate the demands of exercise. These changes occur temporarily during the exercise. Long-term changes also occur in the body metabolism and function.
Previous, Exercise Physiology